Power
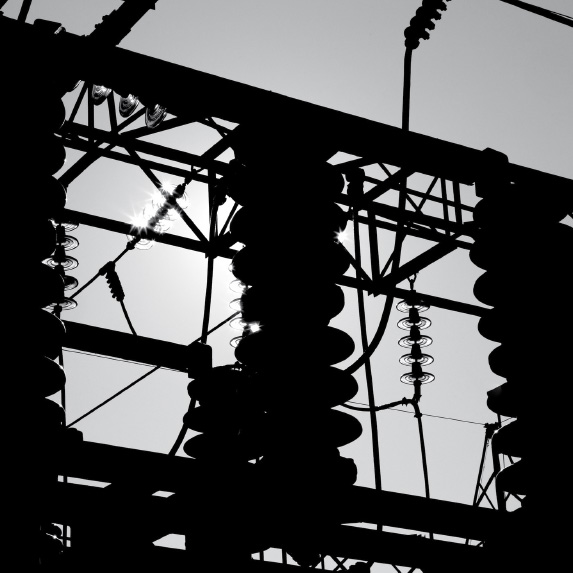
The power sector is already undergoing a decarbonization process in multiple countries around the world. The traditional centralized organization of the power system is now facing a paradigm shift to distributed and renewable generation. This new model is closely related to the implementation of smart grids, where the end users act as prosumers by supplying the network with the excess of power generation produced by their distributed photovoltaic (PV) systems. Digital technologies will be at the center of this revolution, unlocking the potential of different business models like virtual aggregators and peer-to-peer energy trading.
The current technologies supporting this transition can be classified into four main groups:
- low-carbon energy sources (on- and offshore wind, solar PV and concentrated solar power, hydropower, biomass, nuclear, and geothermal)
- short-term and long-term electricity storage solutions
- other flexible options such as network interconnections, sector coupling, supply response (hydro reservoirs, bioenergy) and demand-side management (DSM)
- carbon capture, utilization, and storage (CCUS), and variants including bio-energy CCUS and direct air capture
While many of these technologies are already cost-competitive and may offer even lower costs in the future, others (e.g. electricity storage and carbon capture) require future technological developments and/or increased economies of scale to support their effective deployment at the levels needed to reach a full decarbonization of the power sector.
The total decarbonization target will require a combination of multiple technologies. Depending on local conditions, the mix of available power options will vary from one country to another, and thus there will be no one-size-fits-all solution. The implementation of transition technologies may also be required. Coal should be phased out earliest given its high carbon content and its contributions to air pollution. While natural gas may play a crucial role during a transition period, it will also need to be either decarbonized or progressively phased out. In all cases, countries should prepare detailed plans exploring all options for decarbonization, and their associated costs. To allow for unanticipated technological breakthroughs and cost reductions, energy policies need to be flexible, to be regularly assessed, and be adaptive to ongoing technology advances in order to allow each potential low-carbon solution to be supported and deployed.
While many national and international policies are heavily oriented towards the electrification of energy systems, electrification must proceed alongside decarbonization in order to fight climate change. Also, the energy efficiency potential along the whole electricity chain should not be underestimated. Moreover, a strongly integrated approach across sectors and energy pathways is essential for addressing climate change issues. Finally, secondary effects and a holistic perspective on the entire lifecycle of technological solutions should be considered to avoid potential rebound effects from specific technology choices.
Case Studies
A strong decarbonization of the power sector needs multiple flexibility solutions to support a large integration of variable renewable energy sources. For this reason, this section presents some case studies focused on new flexibility solutions, including battery grid storage, vehicles-to-grid (V2G), power-to-gas, and virtual power plants.
Battery grid storage
Electricity storage has always been challenging at the grid level, and generally the only commercial solution for large-scale and medium- to long-term electricity storage is the use of pumped hydro storage in countries that have compatible geographic conditions. However, the need to integrate non-dispatchable RES is pushing the deployment of batteries at the grid level, with Lit-ion batteries taking the lead, as already discussed in the previous section. While the main challenges and aspects have already been cited, this paragraph aims at presenting some case studies to highlight some technical features of large-size power batteries.
At 100MW/129MWh, the Hornsdale Power Reserve, developed by Tesla, is the largest Li-ion battery in the world, and it provides network security services to the South Australian electricity power grid.[1] The storage system has been in operation since December 2017, and it was built with a challenging timeline to ensure that the battery was available during the 2017 peak demand summer season, after blackouts in the previous year. The system provides frequency control and short-term network security services, and a portion of the battery will also be dedicated to trading on the electricity market. The battery is connected to the Hornsdale Wind Farm, whose excess power output is stored when demand is low and dispatched to the grid when demand is high.
NEC Energy Solutions provided a Li-ion phosphate battery in Maui, Hawaii[2] in a 21 MW wind farm. The battery has an output power of 11 MW and a capacity of 4,300 kWh. It was installed to manage wind farm ramp rates to comply with local interconnection requirements that are in place to ensure local power grid stability by limiting feed-in variability of generating resources. The project was not directly subsidised, but it was part of the wind farm which qualified for a 30% investment tax credit. Overall project costs were mainly related to equipment cost, with the largest part due to the Li-ion cells of the battery. Variable costs are driven by energy losses and auxiliary consumption, mainly due to the thermal management equipment (chillers). The round-trip efficiency of the storage system is about 80% (AC-to-AC), including losses due to power conversion from AC to DC and back to AC, the energy storage cells, busbars, battery management systems, and thermal management systems.
In February 2012, a large-scale utility project was built in Zhangbei, Hebei Province, China, developed by Build Your Dreams (BYD) and the State Grid Corporation of China (SGCC). The installation includes 100 MW of wind power, 40 MW of solar PV, and 36 MWh of Li-ion electricity storage. It is worth mentioning that the batteries used in the system are second-life Li-ion car batteries, which were used before in 36 taxi cars operated by BYD for about 4000 cycles.[3]
Vehicles-to-grid
The Vehicle-to-grid (V2G technology enables a bi-directional energy transfer from/to electric vehicles. This is an evolution of the traditional one-way charging and of the ‘smart’ charging (often referred as V1G), where the rate and time of charge can be varied based on specific objectives (e.g. maximizing the use of available generation from RES). The increase of EV penetration leads to new challenges for the power grid management, but at the same time, additional opportunities can be unlocked by exploiting the storage potential of the EV batteries. While the large majority of the current EV fleet and charging infrastructure is not supporting V2G technology, there are a number of pilot projects worldwide that aim at demonstrating its feasibility and exploring its potential.[4] Some case studies are presented here, to highlight the main challenges and opportunities of V2G.
One of the first deployments of V2G infrastructure has been carried out in the JUMPSmartMaui project, on the island of Maui, Hawaii.[5] The installation of 80 Vehicle-to-home (V2H) chargers was part of a larger project aimed at developing a smart grid with the integration of renewables, EV, energy storage, and controllable loads. The project has been carried out by Hawaiian and Japanese stakeholders and is headed by the New Energy and Industrial Technology Development Organization (NEDO) of Japan. The V2H chargers were used to help manage the evening peak of the power demand (6-9pm), by discharging their batteries in response to grid signals related to distribution system loads and frequency events. The first part of the trial involved two years of V1G operation, to collect reliable data on driving patterns and ease the introduction of the V2G technology. One of the main outcomes of this project has been the EV battery’s ability to provide very fast and flexible services to the grid that can be very valuable when combined with other flexibility resources. Some lessons learned included the importance of focusing on the incentives for the final users towards EV plug-in. In the absence of such incentives, i.e. if the families use their EVs normally, the EVs are not always plugged in at the evening, especially when they can charge them during the day using the public fast charging stations.
The Parker project,[6] developed in Denmark from 2016 to 2018, was the world’s first fully commercial V2G hub. The project has investigated the grid applications that contemporary EVs can provide to power systems, by systematically listing potential power and energy services in a so-called ‘service catalog’. The emphasis has been on frequency regulation services, since they are not only the most demanding (reaction time and need of V2G) but also the most commercially interesting services currently required in Denmark. Among the aims of the project were the comparison of the capability of different cars, as well as the identification of potential barriers to commercialisation. By integrating different vehicle fleets in multiple locations (municipalities, commercial companies, and ports), the project was able to provide a 24/7 service to the grid. The test highlighted the importance of understanding the schedule of each customer, as well as their required state-of-charge at a specific time, in order to optimize the organization of the system. The main barriers that have been found include the long duration of the frequency services required (limited by the small capacities of the batteries), the low efficiency when operating the chargers at power levels lower than the rating, and some battery degradation.
The INVENT project (Intelligent Electric Vehicle Integration),[7] represents a large-scale trial in the UC San Diego campus, with multiple vehicle types and chargers, supporting the move towards commercial deployment in California. The trial is in collaboration with UC San Diego’s Triton Ride Program, which operates a fleet of EVs that safely transport students around campus at night. Thus, the combination of the university fleet charging at night and the workplace charging during the day allows a 24-hour service. The project will test vehicle-to-building (V2B) integration, demand response, frequency regulation, and interaction with solar forecasting in the framework of the UC San Diego microgrid that is also equipped with solar generation and storage. Both AC and DC chargers are tested, with more than six different vehicle types. The main challenges include the availability of cars, the drivers’ varying personal schedules, and the optimization of plug-in times by assigning convenient parking locations to project drivers. Among the first results of the project, there is evidence that V2G does cause some additional battery degradation, but much smaller than that experienced through driving behaviour (and particularly regenerative braking). Potential damage depends on the service, with full charge/discharge cycles being the worst. Car manufacturers may move towards a certification system to become an approved aggregator or charger.
Power-to-gas
The power-to-gas (P2G) technologies represent the solutions available for the generation of a low-carbon synthetic gaseous fuel, either hydrogen of methane, by exploiting the electricity produced from renewable energy sources. Hydrogen generation through electrolysis is commercially available, but it currently represents a very limited share of worldwide hydrogen production due to higher costs in comparison with other processes using natural gas and coal as input resources. In this section, some case studies of P2G systems for the production of synthetic methane based on electricity from renewables are presented.[8]
The largest industrial facility built in the world to produce synthetic natural gas from electricity is the Audi e-gas plant, under operation since 2013 and located in Werlte (Germany), with a nominal rated power of 6 MWe. The technology is based on the catalytic methanation of pure hydrogen and carbon dioxide in a single isothermal fixed- bed reactor. The hydrogen is produced by three alkaline electrolysers connected to an offshore wind park co-financed by Audi AG and a regional power-supply company (four 3.6 MWe turbines). In addition, the flow of CO2 required for methanation is separated from the raw biogas of a neighbouring biomethane plant, by means of amine scrubbing. The entire P2G process has an efficiency of 54% (without accounting the useful heat produced in the process). The maximum output flow of the facility is 325 Nm3/h, but the limited availability of input power (around 4,000 h per year) leads to a production of 1,000 tonnes per year. The P2G plant, in contrast to the biogas plant, is not operated on a continuous basis but rather at variable load following the power supply pattern of the wind park. Furthermore, the plant has been qualified for participating in the electricity balancing market, after successfully drawing 6 MWe of power from the grid within five minutes as well as running prescribed load profiles. The thermal management of the heat recovered from electrolysis and methanation, to supply the various heat consumers in the biogas and CO2 removal plants (mainly amine regeneration), is highly complex.[9]
The HELMETH project (Integrated High-Temperature Electrolysis and Methanation for Effective Power to Gas Conversion)[10] has been co-financed by the European Union’s Seventh Framework Programme and took place from 2014 to 2017, coordinated by the Karlsruhe Institute of Technology (Germany). This project demonstrated the feasibility of a highly efficient P2G technology by thermally integrating high temperature electrolysis (a solid oxide electrolyzer cell (SOEC) technology) with methanation. The demonstration plant’s operations were semi- automated. Although the coupling wasn’t completely successful, an efficiency of the integrated system of about 76% HHV could be achieved.[11] Some technical issues identified within the project are the difficulty of operating at extremely low volume flow rates at the electrolyser inlet, and the performance of insulation materials operating at high temperatures and pressures. According to the promoters, by scaling up the HELMETH concept, an overall P2G efficiency over 80% could be obtained by reducing the parasitic losses. In addition, a scale up techno-economic study was performed in the project, as well as an LCA report of the overall process against benchmark scenarios.[12]
The P2G-BioCat project,[13] developed in Denmark from 2014 to 2016, had the objective of designing, engineering, constructing, and operating a 1-MW power-to-gas facility to produce and inject grid-quality methane under intermittent operations using hydrogen from alkaline electrolysis and methanation reactor based on biological catalyst. The project’s technical and business goals have largely been achieved, providing useful elements for the commercial development of biological methanation and P2G for the benefit of the Danish energy system. The system operated for 8 months during the project,[14] with a total consumption of 42,193 Nm3 of biogas, 170 m3 of water, and 708,215 kWh of electricity for the system operations, to produce 129,290 Nm3 of hydrogen making available ~15,000 Nm3 of renewable methane and recovering 85,000 kWh of heat for use at a nearby facility.
Power-to-liquids
Power-to-liquid (P2L) technologies represent solutions available for the generation of low-carbon intensity synthetic liquid fuel, such as methanol, by CCU and electrolysis. The largest industrial facility to produce a liquid fuel from electricity is Carbon Recycling International’s (CRI) renewable methanol plant in Svartsengi, Iceland, under operation since 2011.[15] The plant captures CO2 from power-plant flue gases by means of amine scrubbing and utilizes three alkaline electrolyzers with a rated capacity of 6 MW electric to produce up to 12 tons of methanol per day. The output of this plant has been used by clients for gasoline blending, under EU standards, as a neat fuel (M100) for internal combustion engine vehicles as well as direct methanol fuel cell vehicles, biodiesel production, water purification and various chemical products, thus demonstrating the diverse uses of the low-carbon intensity methanol in both fuel and chemical applications.
Virtual power plants
Virtual power plants (VPP), also known as virtual aggregators, are made by exploiting digital technologies to coordinate power generators, end users, and storage systems with the aim of optimizing the aggregated load based on the evolution of market prices, or to provide ancillary services to the power grid. VPPs are often implemented to increase the profitability of distributed generation from RES, by providing to small players access to pools and markets that generally have a limiting threshold on the minimum capacity. Some VPPs are commercially implemented in Europe, the USA, and Australia.
One of the biggest VPPs in Europe is operated by Next Kraftwerke,[16] which spans its energy network all over Germany and has begun to implement VPP in other countries (Austria, Belgium, France, the Netherlands, Poland, Switzerland, and Italy). As of March 2019, more than 7,500 units are connected to the system, for a total capacity reaching almost 6.9 GW, resulting in around 12 TWh of electricity traded annually. Different power-producing assets are connected to a central control platform, from renewable sources such as biogas, wind, and solar, to commercial and industrial power consumers and electricity storage systems. Kraftwerke, which has started its activities in 2009, has also specialized in short-term dispatch and trading.
Another interesting case study, although in an earlier stage of maturity, is being deployed in South Australia. Tesla is working with the South Australian Government and a local electricity retailer to create a VPP that could eventually include up to 50,000 households.[17] The VPP, which is being introduced as a trial with three phases, is currently in the second phase, after the phase was successfully tested on 100 households. After the completion of Phase II, 1,000 households will have home electricity storage systems installed, and another 320 households will share in the benefits of the VPP as program participants. If the Phase II trial meets its objectives and obtains private financing, Phase III could start scaling up to an additional 49,000 households from mid-2019. Operating at full scale, the VPP could generate 250MW and store 650MWh. At full scale the VPP will make electricity more affordable and reliable across the state by introducing competition to the market, adding new dispatchable supply, and boosting the security of the network by increasing the role of existing renewable assets.
Carbon capture and utilization
Carbon capture and utilization (CCU), sometimes also referred to as carbon-to-value, includes the multiple technologies that allow recylcing of the CO2 stream obtained by flue gases or air to manufacture a range of products, including cement, carbonates, chemicals, plastics, fuels, etc. The aim of these processes is to find an alternative to CCS, by overcoming the concept of burying carbon emissions underground and instead providing effective value by creating products that would have consumed other resources for their production. In some cases, e.g. when producing fuels, the carbon dioxide may be released again into the atmosphere, but the entire cycle will become (almost) carbon neutral, if no fossil CO2 is involved. There is a growing interest in CCU applications worldwide, and some companies are already providing commercially-competitive solutions, although often at a limited scale. As a result, the case studies presented below generally have a lower maturity in comparison with the ones illustrated in the previous paragraphs.
Mineral Carbonation International (MCi),[18] an Australian based privately-owned company, is developing a technology platform for large scale transformation of CO2 into magnesium carbonate, which can be used to produce cement, paving stones, and plasterboard. The CO2 is combined with minerals or waste streams through an accelerated natural process called mineral carbonation. In July 2018 the company completed a 5-year research pilot plant program funded by Australian Government and Industry, reaching a solid techno/economic validation of the technology. If successful at scale, this would assist as a transition technology for the major emitting countries as they move away from carbon intensive industry to more sustainable energy mixes and low carbon economies. The current pilot plant is at the basis for a demonstration plant that could process 5,000-10,000 tonnes per annum of CO2, planned in the next three years. This would then scale roughly every three years to a further 10-fold scale increase in capacity, the final target scale being 1 million tonnes of CO2 produced per plant.
Founded in 2003, after over a decade of research, Newlight,[19] based near Los Angeles, California, has developed a carbon capture technology able to produce high-performance bioplastics from carbon emissions. This technology converts greenhouse gases into high-performance biodegradable plastic replacement called AirCarbon®, which is estimated to have the ability to out-compete fossil-fuel based plastics globally both on sustainability and price basis.[20] This biopolymer is made by combining air with methane or carbon dioxide greenhouse gas emissions, resulting in a material that is approximately 40% oxygen from air and 60% carbon and hydrogen from captured carbon emissions by weight. The technology is at an early commercial stage and the materials produced by the company have already been commercialized or adopted for commercialization programs by multiple companies worldwide.
Another interesting CCU application is related to the concrete industry, which is responsible for a significant amount of carbon emissions worldwide. CarbonCure is a Canadian company that developed a technology which uses recycled CO2 to improve the manufacturing process of concrete, with nearly 100 installations in concrete plants across North America.[21] Their process exploits existing production equipment to produce, in situ, a nano-sized mineral carbonate embedded within the concrete by using the recycled CO2 stream without impacting normal plant operations. In a process known as CO2 mineralization, the carbon dioxide is converted to a mineral and it is permanently captured, improving the compressive strength of the concrete.
“Hornsdale Power Reserve.”Hornsdale Power Reserve. Accessed August 20, 2019. https://hornsdalepowerreserve.com.au/ ↩︎
IRENA. “Case Studies: Battery Storage.” IRENA. Accessed August 20, 2019.
https://www.irena.org/documentdownloads/publications/irena_battery_storage_case_studies_2015.pdf ↩︎Jäger-Waldau, Arnulf. “PV Status Report 2014.” JRC. Published 2014. Accessed August 20, 2019. http://publications.jrc.ec.europa.eu/repository/bitstream/JRC92477/pv status report 2014 online.pdf ↩︎
Everoze & EVConsult. “V2G Global Roadtrip: Around the world in 50 projects.” Published 2018. Accessed August 20, 2019. http://parker-project.com/wp-content/uploads/2018/12/VGI-Summit-Day-2-S1-Initiatives-UKPN001-S-02-B.pdf ↩︎
Irie, Hiroshi. “NEDO Smart Community Case Study.” NEDO. Accessed August 20, 2019. https://www.nedo.go.jp/content/100864936.pdf ↩︎
“Parker.” Parker. Last modified 2016. Accessed on August 20, 2019. https://parker-project.com/ ↩︎
Nuvue. “UCSD Invent.” Nuvue. Last modified 2019. Accessed on August 21, 2019. https://nuvve.com/projects/ucsd-invent/ ↩︎
Bailera, Manuel, Pilar Lisbona, Luis M. Romeo and Sergio Espatolero. “Power to Gas projects review: Lab, pilot and demo plants for storing renewable energy and CO2, Renewable and Sustainable Energy Reviews.” Renewable and Sustainable Energy Reviews 69, (2017): 292-312. https://doi.org/10.1016/j.rser.2016.11.130 ↩︎
Ibid., 292-312. ↩︎
Helmeth. “Integrated High-Temperature Electrolysis and Methanation for Effective Power to Gas Conversion.” Helmeth. Accessed August 21, 2019. http://www.helmeth.eu/index.php/project ↩︎
Helmeth. “Helmeth Periodic Report No 2.” Helmeth. Accessed August 21, 2019. http://www.helmeth.eu/images/joomlaplates/documents/Publishable_summary.pdf ↩︎
Helmeth. “Deliverable 5.2: Final LCA Report.” Helmeth. Accessed August 21, 2019. http://www.helmeth.eu/images/joomlaplates/documents/HELMETH_DELIVERABLE_5.2_R1.0.pdf ↩︎
BioCatProject. “BioCatProject - Power-To-Gas Via Biological Catalysis.” BioCatProject. Accessed August 21, 2019. http://biocat-project.com/ ↩︎
BioCatProject. “Final Report.” Energiforskning. Accessed August 21, 2019.
https://energiforskning.dk/sites/energiteknologi.dk/files/slutrapporter/12164_final_report_p2g_biocat.pdf ↩︎Carbon Recycling International. “The George Olah Renewable Methanol Plant.” Carbon Recycling International. Accessed August 21, 2019. https://www.carbonrecycling.is/george-olah ↩︎
“Next.” Next. Accessed on August 21, 2019. https://www.next-kraftwerke.com/ ↩︎
Government of South Australia. “South Australia’s Virtual Power Plant.” Government of South Australia. Last modified 2019. Accessed August 23, 2019. https://virtualpowerplant.sa.gov.au/ ↩︎
“Mineral Carbonation International.” Mineral Carbonation International. Last modified 2019. https://www.mineralcarbonation.com/ ↩︎
Newlight Technologies, Inc. “Newlight.” Last modified 2019. https://www.newlight.com/ ↩︎
XPRIZE. “Transforming CO2 Into Valuable Products.” XPRIZE. Last modified 2019. https://carbon.xprize.org/prizes/carbon ↩︎
Carboncure. “From Carbon to Simply Better Concrete.” Carboncure. Last modified 2019. https://www.carboncure.com/ ↩︎